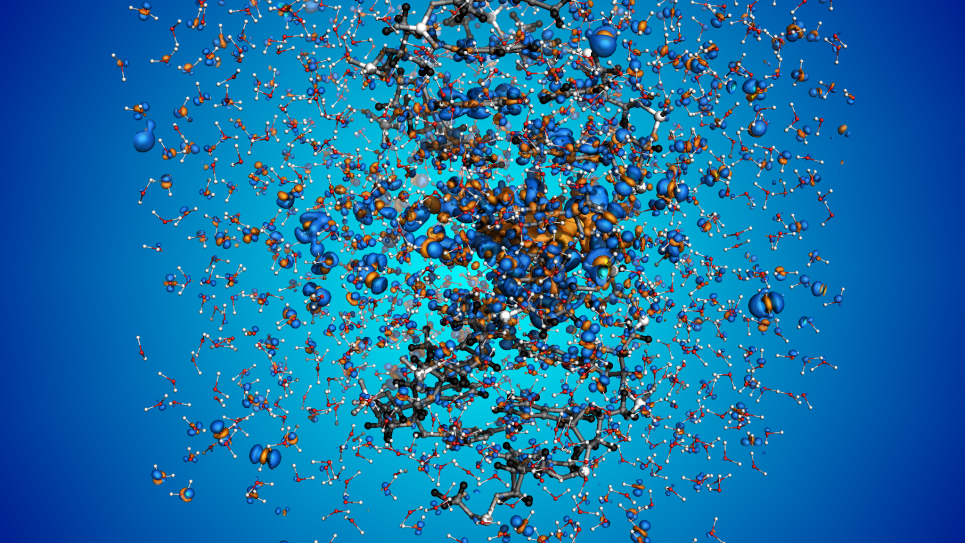
The question of what happens at the microscopic scale during electronic stopping processes—that is, what happens when kinetic energy is transferred from charged particles, such as protons, to the electrons in a target material—drives the advancement of multiple modern technologies: focused-ion beam fabrication, proton-beam cancer therapy, and even nuclear reactor material design all depend on the phenomenon’s elucidation.
The physics governing electronic stopping is the quantum-mechanical excitation of electrons as induced by highly energetic ions, the predictive modeling of which has been hindered for many decades due to the difficulties inherent in describing such behavior. Recent innovations in first-principles calculation methodologies and massively parallel supercomputing, however, have enabled atomistic-level simulations of these processes that achieve unprecedented accuracy. As such, a team of researchers from University of North Carolina at Chapel Hill (UNC), University of Illinois at Urbana-Champaign (UIUC), and Lawrence Livermore National Laboratory (LLNL) is leveraging computing resources at the Argonne Leadership Computing Facility (ALCF)—a U.S. Department of Energy (DOE) Office of Science User Facility—to run a series of large-scale simulations so as to gain further insight into electronic stopping.
Led by UNC’s Yosuke Kanai, the work is pertinent to any situation in which matter is exposed to radiation—standing to illuminate, for instance, what happens to a patient’s genetic material during therapies to treat cancer. With ALCF computing time awarded through DOE’s Innovative and Novel Computational Impact on Theory and Experiment (INCITE) program, the team’s simulations are providing a level of detail that improves overall computational predictive power while also answering critical questions such as how much energy is required to fragment DNA.
Kanai grew interested in electronic stopping while working at LLNL, where he was developing real-time time-dependent density functional theory (RT-TDDFT)—a quantum-mechanical modeling method—for large-scale implementation. Electronic stopping, given its ubiquity, is critical for various DOE missions and seemed an ideal problem to investigate through the use of his new simulation capabilities.
The team’s initial modeling efforts at the ALCF concerned condensed matter systems (systems comprising an extremely
large number of strongly interacting elements). The facility’s supercomputers enabled the researchers to carry out high-resolution simulations of the irradiation of magnesium oxide with silicon projectiles, uncovering new details about the role of core-electron excitation and transfer.
Efforts to improve the accuracy and stability of the simulations have seen collaborators at UIUC further develop the relevant mathematics (a problem of coupled nonlinear partial differential equations), while Kanai’s group at UNC has been working to refine the key approximation of RT-TDDFT: the so-called exchange-correlation approximation, which describes quantum-mechanical electron interactions. The ALCF Computational Performance Workshop provided the opportunity for two of the team’s researchers, PhD students Cheng-Wei Lee of UIUC and Dillon Yost of UNC, to work side by side with ALCF staff to optimize the project’s code, for which effective use of two parallelization schemes is crucial.
“Efficient massively parallel implementation is necessary for our project’s success given our need to simulate the quantum-mechanical dynamics of electrons in large, complicated systems,” Kanai said. “We are grateful for the INCITE award because this work could not be performed without accessing leadership-scale computing resources like Theta and Mira.” Indeed, some of the simulations model more than 13,000 electrons—conditions that require hundreds of thousands of processors to operate in parallel.
UNC’s prominent medical school, along with the ample number of biochemistry and biophysics researchers on campus, motivated the team to transition from studying solid materials such as silicon to genetic matter like DNA. “When I learned of proton beam therapy, which is a promising radiation oncology technique, I realized that the physics making it work is the same as what we study at the quantum-mechanical level when we investigate the radiation damage of solids,” Kanai recalled. “The only differences are terminological.”
Kanai’s team simulates irradiation of both dry and solvated DNA, the latter being especially computationally expensive.
In perhaps their most significant results to date (published in Journal of the American Chemical Society), the team’s simulations unraveled complicated details about the electronic excitation behavior of DNA under proton and alpha-particle irradiation, employing methods that yielded a molecular-level understanding of the involved processes. Excited states induced by light were compared against those induced by silicon projectiles: photon-based radiation (such as x-rays and gamma rays) primarily excited core and semi-core electrons, while the silicon projectiles tended to excite valence electrons. “These findings are not only scientifically interesting, they’re also extremely important in broader contexts—particularly that of radiation oncology,” Kanai explained.
The first-principles approach embraced by Kanai has permitted a relatively seamless transition. “While the scientific issues underlying the various settings differ, we employ the same RT-TDDFT method in treating each case without the need to tune empirical parameters for a specific system,” Kanai elaborated. The differences in the underlying considerations are immediate: In the context of modeling radiation damage, solid-state systems such as magnesium oxide and silicon under high-Z ion irradiation (that is, irradiation with ions featuring a large number of protons in their nuclei) depend heavily on the charge state of the projectiles. If one studies DNA in the context of radiation oncology, on the other hand, the question of how electronic excitation under proton irradiation contrasts with that under typical photo irradiation will inevitably arise. RT-TDDFT has proved to be a powerful tool for examining each case.
Validation of simulation results is a priority for the DNA component, as comparisons can be made with data obtained from spectroscopic studies of dry genetic material. But, as Kanai noted, a principal question to be resolved is how the behavior of water-solvated DNA—which is the more relevant condition in the context of proton beam therapy—differs from the available empirical data about the dry case. “We’ve already made substantial progress in understanding what distinguishes irradiation of solvated DNA from dry DNA, but we still have a long way to go,” he said. His team also plans to examine how excitation behavior changes when a target is irradiated with carbon ions: carbon ions, it has been suggested, might prove more effective than protons in radiation oncology—which could revolutionize this important field of medicine.
Argonne National Laboratory seeks solutions to pressing national problems in science and technology. The nation's first national laboratory, Argonne conducts leading-edge basic and applied scientific research in virtually every scientific discipline. Argonne researchers work closely with researchers from hundreds of companies, universities, and federal, state and municipal agencies to help them solve their specific problems, advance America's scientific leadership and prepare the nation for a better future. With employees from more than 60 nations, Argonne is managed by UChicago Argonne, LLC for the U.S. Department of Energy's Office of Science.
The U.S. Department of Energy's Office of Science is the single largest supporter of basic research in the physical sciences in the United States and is working to address some of the most pressing challenges of our time. For more information, visit https://energy.gov/science